Nutrients Removal and Recovery in Bioelectrochemical Systems a Review
Abstract
Conventional wastewater purification technologies eat large amounts of energy, while the arable chemical energy and nutrient resources contained in sewage are wasted in such handling processes. A microbial nutrient recovery cell (MNRC) has been adult to accept advantage of the energy contained in wastewater, in order to simultaneously purify wastewater and recover nutrient ions. When wastewater was circulated between the anode and cathode chambers of the MNRC, the organics (COD) were removed by leaner while ammonium and phosphate (NHfour +-N and POfour three−-P) were recovered by the electrical field that was produced using in situ energy in the wastewater without additional free energy input. The removal efficiencies from wastewater were >82% for COD, >96% for NH4 +-North and >64% for POfour 3−-P in all the operational cycles. Simultaneously, the concentrations of NH4 + and PO4 three− in the recovery bedchamber increased to more than 1.5 and 2.ii times, respectively, compared with the initial concentrations in wastewater. The MNRC provides proof-of-concept as a sustainable, self-driven approach to efficient wastewater purification and nutrient recovery in a comprehensive bioelectrochemical system.
Introduction
Global water shortages and resources crises acquired by population explosion have become crucial concerns for the evolution of human guild over the course of several decades. Researchers accept focused on exploring new sources of water, free energy and nutrients; nevertheless, reusing water and recovering resource and free energy from wastewater are fifty-fifty more than of import and sustainable, since wastewater contains abundant nutrients and chemical energy as well equally providing a reusable h2o resourceone. Conventional wastewater treatments have mainly focused on purification rather than resource recovery. The near ordinarily used process, aerobic/anoxic/aerobic (A2O) procedure, is mainly utilized for removal of COD and nutrients merely not their recovery. Conventional treatment technologies therefore waste material the nutrients and chemic free energy independent in wastewater, while simultaneously consuming large amounts of electricity in the aeration procedure. Researchers have fabricated nifty efforts to explore feasible technologies in attempts to recover resources and free energy independent in wastewater. It was reported that adsorption using several kinds of sorbents could separate phosphate from wastewaterii. Ion commutation, which acted as a reversible process, could be used to recover both ammonium and phosphate3,iv. A mutual and applied arroyo is to simultaneously recover ammonium and phosphate as struvite from sludge digestion tanks where phosphorus is released to liquor from phosphorous enriched biosolids discharged from municipal wastewater handling plants5,6,vii. Enrichment of phosphorus from wastewater into activated sludge could be achieved by the grouping of polyphosphate-accumulation organisms (PAOs) through alternant anaerobic and aerobic weather. However, this biological enrichment process is usually associated with energy consumption. Therefore, it would be significant if the nutrient could be cost-effectively and directly recovered from wastewater without additional free energy input.
Bioelectrochemical systems (BESs) are a novel wastewater handling technology that can oxidize substances in wastewater and simultaneously generate electricity or produce valuable compounds using bioelectrically agile bacteria8,nine,10,eleven,12,xiii. Typical BESs include microbial fuel cells (MFCs) that generate electricity14,15,16,17,eighteen, microbial electrolysis cells (MECs) that produce hydrogenxix,xx,21 and microbial desalination cells (MDCs) that desalinate brackish water using pairs of cation and anion commutation membranes22,23,24,25,26. The emergence of MDC represents a new field in the use of electric ability derived from the free energy in wastewater to realize direct migration of charged ions and thus water desalination. It has revealed the possibility that nutrients in ionic forms in wastewater could also be driven across membranes within a BES. Several studies of nutrient removal via BES showed the potential for this application27. The BESs based on the MEC configuration which achieved nutrient removal with hydrogen production showed a nifty removal functioning when an appropriate external voltage was applied28,29,30. A newly reported approach, termed R2-BES, attempts to recover nutrients from wastewater31. Ammoniums were migrated out of the wastewater by the driving force of bioelectricity while phosphates were exchanged with hydroxyls generated in a cathode reaction. The R2-BES could achieve the desired issue with advisable external voltages practical.
Inspired past the concept of MDC, a newly developed technology, termed a microbial nutrient recovery cell (MNRC) is presented hither, for simultaneous efficient removal of COD and nutrients too equally in situ recovery of ammonium and phosphate ions, driven by the energy in wastewater. In the MNRC, a cation exchange membrane is positioned near the anode chamber and an anion commutation membrane is positioned near the cathode bedchamber, thus forming a recovery bedroom between the membranes. When wastewater is recirculated between the anode and cathode, the ammonium ions and phosphate ions are pushed into the recovery sleeping room from the anode and cathode sides, respectively, by the driving force of bioelectricity generated from wastewater. The removal performance of COD, ammonium and phosphate were examined and the recovery process was investigated in particular to reveal the extent of nutrient concentration and ion migration contest in the MNRC.
Results
MNRC operation and pollutant removal
The duplicated MNRC reactors each consisted of iii chambers: an anode sleeping room, a recovery bedroom and a cathode bedroom (Fig. 1). The constructed wastewater, which acted as the electrolyte, was recirculated betwixt the anode and cathode. The recovery solution, which received the food ions migrated from the wastewater, was circulated individually. The MNRC effectively reduced the concentrations of COD, NHiv +-Due north and PO4 3−-P in the synthetic wastewater over a 24 h operation cycle (Fig. 2A). The effluent contained COD < 37 mg/50, NH4 +-Due north < 0.6 mg/L and PO4 3−-P < 2.2 mg/L when the MNRCs were operated with different concentrations of recovery solutions (0, 164 and 328 mg/50 NaCl solution). Removals of these pollutants were >90% for COD, >97% for NH4 +-N and >64% for POfour 3−-P in each experiment (Fig. 2B). These results confirm that synthetic wastewater could be well purified in the MNRC when the initial concentrations of recovery solutions varied within a certain range. However, removal performance all the same showed disparities among the three recovery solution concentrations. The highest removals of COD (92%), NH4 +-N (99%) and POfour three−-P (71%) were simultaneously achieved with recovery solution containing 164 mg/L NaCl. In that experiment, COD was reduced from 369 mg/L to thirty mg/50, NH4 +-Due north was reduced from 23.8 mg/L to 0.3 mg/L and POiv three−-P was reduced from 6.iv mg/L to 1.viii mg/L. The differing removal results for different recovery solution concentrations might be caused past a balance between the MNRC internal resistance and ion diffusions in the recovery chamber. When the concentration of the recovery solution was increased, the conductivity was college, leading to lower internal resistance and higher current production. However, with more concentrated recovery solution, the diffusion of Na+ and Cl− from recovery sleeping accommodation to electrode chambers would be reinforced, resulting in an opposed electric current menstruation and thus a detrimental effect on the overall electric current production. Therefore, the concentration of recovery solution could be optimized to obtain the maximum current, which would pb to optimal contaminant removal efficiency. The concentrations of Na+ and Cl− in the 164 mg/L NaCl recovery solution were similar to those in the synthetic wastewater and thus the undesired diffusion of ions could exist minimized and the highest COD, NH4 +-North and POiv iii−-P removals were achieved.
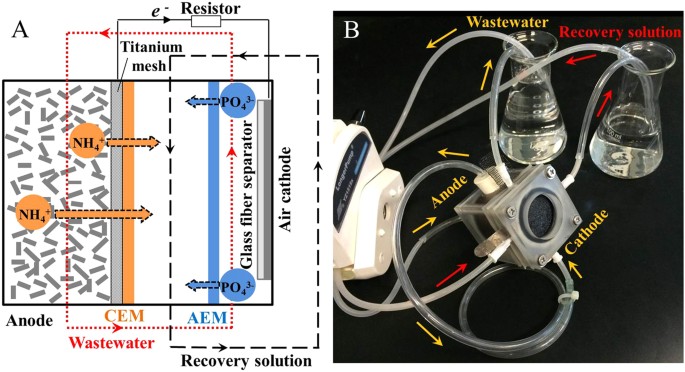
Schematic (A) and photograph (B) of microbial food recovery cell (MNRC); AEM: anion commutation membrane; CEM: cation commutation membrane.
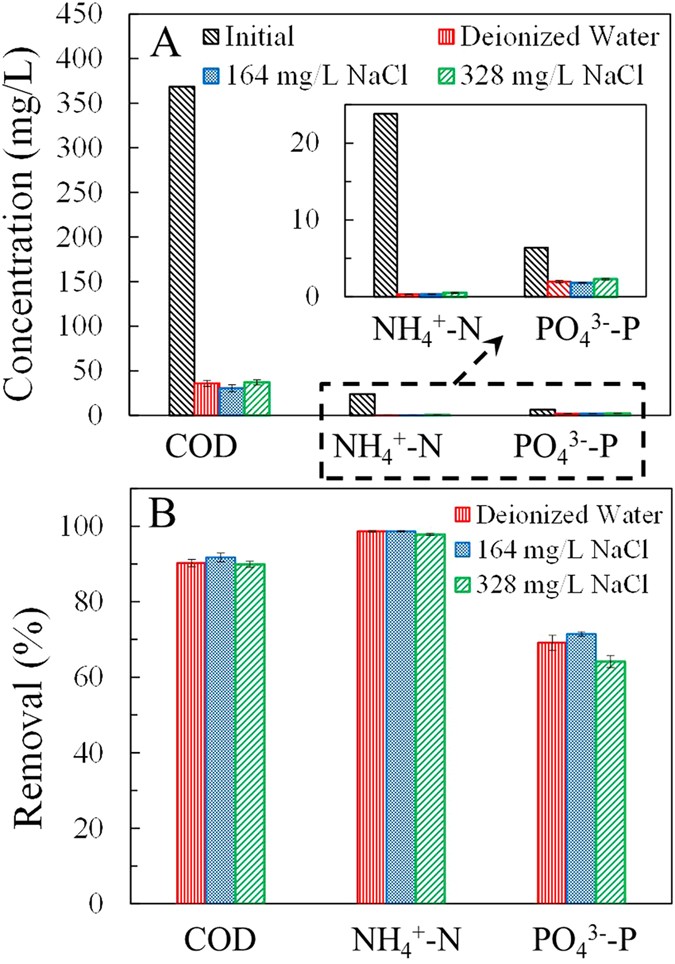
Concentrations in initial and treated synthetic wastewater (A) and removals (B) of COD, NHfour +-Northward and PO4 iii−-P, using recovery solutions containing 0, 164 and 328 mg/L NaCl in a 24 h operational cycle.
Concentrating NH4 +-Northward and POfour 3−-P in the recovery chamber
NH4 +-N and PO4 three−-P were effectively full-bodied in the recovery solution after the MNRC was operated for a whole concentrating examination of 120 h, consisting of five operational cycles (Fig. 3A). The concentrations of NH4 +-N in the recovery solution increased from 0 to 35.seven mg/L and 37.eight mg/L at the end of the beginning and 2nd concentrating tests respectively. Every bit the initial concentration of NH4 +-Due north in synthetic wastewater was 23.8 mg/L, these results betoken that NHiv +-N could exist driven into the recovery solution and concentrated to 1.v times. Meanwhile, POfour 3−-P concentration in the recovery solution reached 16.0 and 14.ii mg/50 at the stop of the offset and the 2nd concentrating tests, which were 2.5 and 2.two times the initial concentration in the synthetic wastewater (6.4 mg/L). COD concentrations in recovery solutions were measured as less than detection limit, which confirms that the ion exchange membranes blocked the nearly of organics in the electrode chambers from polluting the recovery solution. These results demonstrate that the MNRC could collect and concentrate NHiv +-N and POfour 3−-P simultaneously in the recovery solution without COD contamination detected.
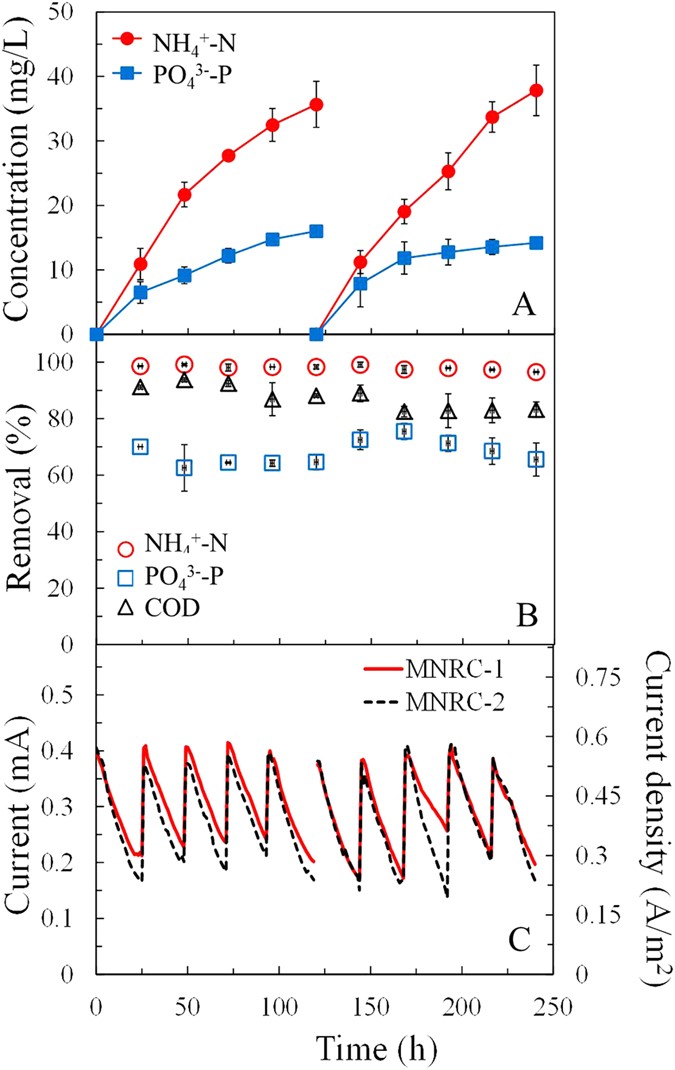
Concentrations of NH4 +-N and PO4 3−-P in the recovery solution (A), removals of COD, NHfour +-N and PO4 three−-P in wastewater (B) and electric current generation of the duplicated MNRCs (refer to every bit MNRC-1 and MNRC-2) (C) in two repeated concentration tests.
The slopes of the concentration curves for NH4 +-N and POfour three−-P decreased during the concentrating test, indicating a slowing of ion recovery. The recovery procedure was driven by electrical power and obstructed past the diffusion of ions from the recovery chamber to the electrode chambers (opposite migration management to the recovery process). The current output performance was repeatable in each operational cycle, demonstrating that the driving force remained relatively consistent during the concentrating tests (Fig. 3C). When fed with fresh synthetic wastewater with sufficient substrate (COD) and neutral pH, the MNRC could generate a reproducible maximum current of ~0.4 mA (0.56 A/k2). The stable COD removal efficiency for each operational cycle was consistent with repeatable current producing operation (Fig. 3B). The pH changes of the electrolyte were also repeatable for each operational wheel, decreasing from vi.8 to ~4.0 (Supporting Data, Fig. S1). Equally the current generation was repeatable, diffusion is likely to be the main cistron leading to the deceleration of nutrient recovery. When nutrient ions accumulated in the recovery sleeping accommodation, the diffusion of ions driven by increased concentration gradients across membranes partially start the recovery process. Other kinds of ions including Na+, H+, Cl− and SO4 2− kept migrating from electrode chambers to the recovery chamber and supplemented the function of nutrient ions to conduct internal current (information and analysis was shown in the post-obit section). Maximum concentration was achieved when the driving force of the electrical field equaled that of the concentration gradients of food ions across the membranes. To achieve college nutrient concentration in the recovery chamber, the MNRC current should be promoted, for case past using wastewater containing college COD, enlarging the reactor or improving the construction of the reactor, such equally using thinner chambers to reduce the solution resistance.
Removal efficiencies for COD, NHfour +-N and PO4 3−-P were >82%, >96% and >64%, respectively, in all the 10 operational cycles during the ii concentrating tests (Fig. 3B). This indicates that the continuous recovery of NH4 +-Ns and PO4 three−-Ps in the recovery solution facilitated steady removal from the synthetic wastewater. The average total removal quantity of POfour three−-P in the two concentrating tests was 1.nine ± 0.one mg, with total recovery of one.2 ± 0.1 mg. This result shows that approximately 63% of the removed PO4 3−-P was recovered in the recovery solution. The removal functioning of NH4 +-North remained high throughout the concentrating tests (Fig. 3B). The average total removal and recovery quantities of NHiv +-N in the two concentrating tests were 11.6 ± 0.1 mg and 2.8 ± 0.1 mg. This indicates that the NHfour +-N that diffused into the recovery solution, driven by the electrical field, merely accounted for 24% of the total removed quantity, whereas more 3 quarters of the NHiv +-N was removed by other paths. Anion-commutation chromatography showed that concentrations of NO3 − and NOtwo − were beneath detectable limits and therefore the presence of these ions could not exist confirmed. The effect of abiotic command shows that, the concentrations of NHiv +-N and PO4 3−-P in the wastewater effluent were nearly the same as that in the initial wastewater (Supporting Information, Fig. S2). In contrast, the result of open circuit control experiment shows that, fifty-fifty without current product, about 60% of NHfour +-N and xvi% of POfour 3−-P were removed. It was probably via bio-uptake of microorganisms in the electrode chambers and circulation tubes of MNRC, concrete adsorption, chemical precipitation, or membrane fouling, etc. In add-on, the nitrification and denitrification might take identify in the cathode bedchamber and thus outcome in the loss of the nitrogen. The overall mass balances and mechanisms in nutrient removal will be further investigated in the hereafter to illustrate the path to improve the performance of this system.
Component changes and charge transfer in the MNRC
The concentrations of all types of ions, except H+, in the synthetic wastewater were reduced subsequently the MNRC completed ane operational bicycle (Fig. 4A). Cations in the wastewater at 0 h included 1.70 mM NHiv +, 1.22 mM Na+ and ii × x−4 mM H+ (according to the pH, 6.8). Later 24 h operation, 1.67 mM NHiv + and 0.66 mM Na+ were removed, while 0.one mM H+ was gained to balance electric charge. Anion tests showed 1.97 mM Cl−, 0.20 mM orthophosphate (referred to as POiv 3−) and 0.32 mM Sofour ii− in the initial wastewater, with 0.57 mM (Cl−), 0.06 mM (PO4 iii−) and 0.05 mM (And soiv 2−) remaining after 24 h. At both the commencement and end of an operational bicycle, the full electric charges of cations equaled those of anions in the synthetic wastewater. These results signal that the MNRC could remove NH4 +, PO4 iii− and other ions from the wastewater. In addition to the removal of NH4 + (98%) and PO4 3 (70%) discussed in a higher place, 54% of Na+, 71% of Cl− and 84% of And so4 2− were also removed from the synthetic wastewater.
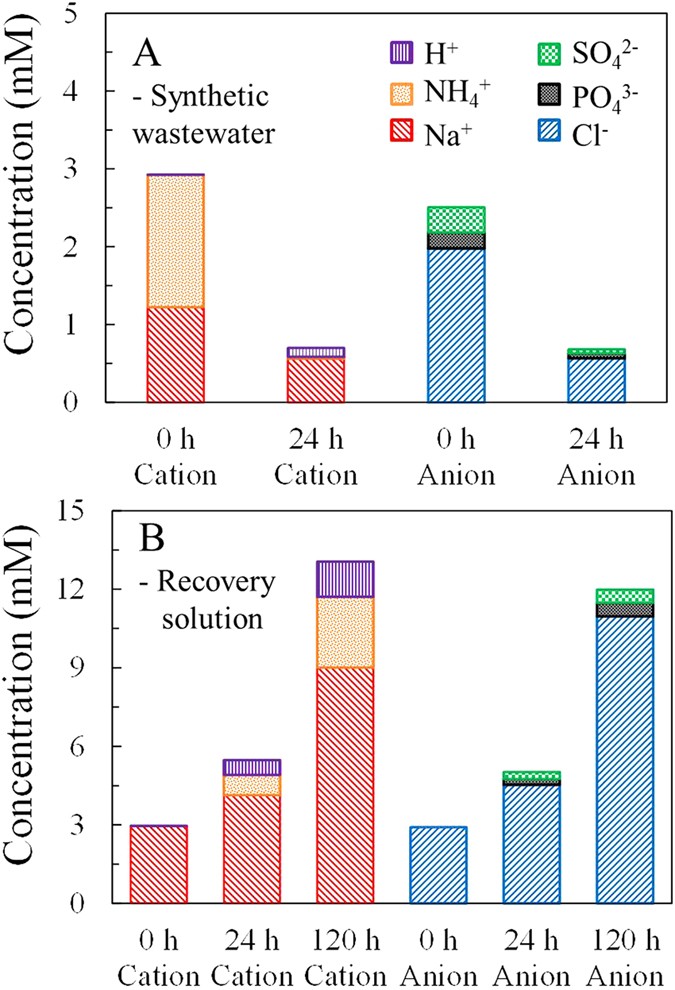
Ion distributions in wastewater at the outset and end of the first operational wheel during a concentrating test (A) and ion distributions in the recovery solution at the offset and end of the outset operational cycle and the end of the concentrating test (B).
Concentrations of all types of ions in the recovery solution kept increasing throughout the 120 h concentrating test (Fig. 4B). After the initial 24 h (i.e., at the end of the first operational wheel), the concentrations of cations increased by i.eighteen mM for Na+, 0.78 mM for NHiv + and 0.74 mM for H+, whereas those of anions increased past 1.63 mM for Cl−, 0.21 mM for PO4 3− and 0.27 mM for SO4 2− in the recovery solution. These results showed that the migration of Na+ was quicker than that of NH4 + and the competitive migration order of anions was Cl− > PO4 3− > And then4 ii− under the conditions used in this experiment. H+ was considered to rest the electrical charge and its concentration changes are not discussed further here. After 120 h the full recovered quantities of those ions were 6.04 mM Na+, two.lxx mM NH4 +, i.35 mM H+, 8.06 mM Cl−, 0.52 mM POiv 3− and 0.49 mM And sofour 2− in the recovery solution. The increments of Na+ and Cl− at 120 h were v.i and 4.9 times those in the first 24 h, demonstrating that these 2 ions maintained a steady charge per unit of migration from synthetic wastewater to the recovery solution throughout the concentrating examination. However, the final concentrations of NH4 +, POfour 3− and Then4 2− were 3.five, ii.5 and i.ix times those in the first operational cycle, which indicates that the migration rates of those ions decreased in the latter function of the concentrating test. The molar electrical conductivity, radius, mobility and concentration of each ion, in combination with the electrical field intensity and exchange selectivity of membrane, were factors affecting migration processes in the solutions and across the membranes32,33. Further studies will be conducted to ameliorate understand the competitive migration characteristics. It should be mentioned that ion recovery quantities were less than removal quantities for all types of ions examined in the MNRC, with losses of: xi% Na+, 77% NHiv +, 42% Cl−, 27% POfour three− and 51% SO4 2−. Those ion losses might be due to bio-uptake, adsorption, precipitation, membrane fouling, or measurement error, etc. The mechanisms of the ion losses will be farther investigated in the future.
Accuse demands analysis of ions in the MNRC
Different kinds of ions along with NH4 +-N and POfour 3−-P in the MNRC were transferred through ion exchange membranes (Fig. 5). Full charge need ratios were l% for cations and 49.ii% for anions, indicating that the charge transfer efficiency of the MNRC was 99.two%. The migration of NH4 + required 15.v% of the full accuse, which was less than that of Na+ (23.4 %) and higher than that of H+ (eleven.1%). Meanwhile, the charge need ratio of PO4 3− was 6.three%, which was the everyman compared with those of 32.3% for Cl− and 10.half dozen% for SOiv 2−. Na+ and Cl− each occupied the highest electrical transfer capacity of the MNRC amid all the cations and anions. The results are attributed to the combined effects of ionic characteristics (such as size, accuse and concentration), electrical field and membrane substitution selectivity, as discussed in the previous section. Thus, the competition betwixt desired ions (NH4 + and PO4 three−) and other ions will be further investigated to obtain college recovery performance. Additionally, the transfer of H+, which was used to balance solution pH, also required remarkable electrical charges and thus constructive methods of stabilizing acidity might promote the collection of NH4 +.
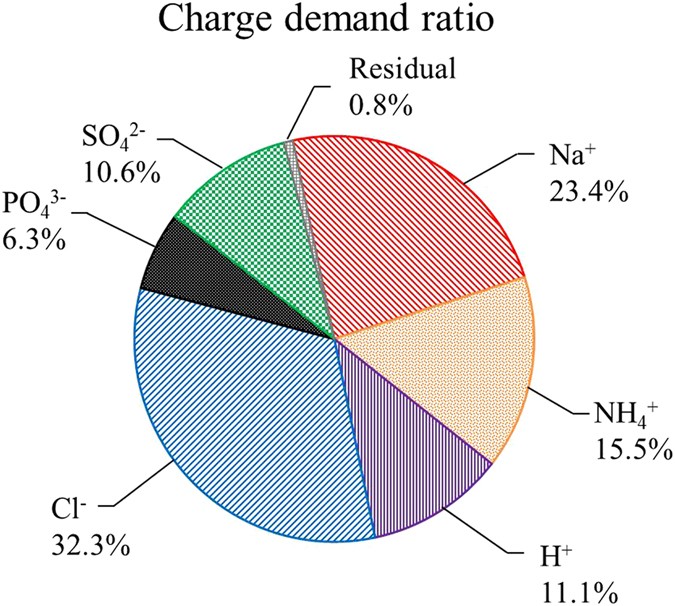
Charge demand ratio of each blazon of ion in the recovery solution during a 24 h operational cycle.
Discussion
The MNRC adult in this report is a proof-of-concept that might enable simultaneous wastewater purification and nutrient recovery without additional free energy input. Concentrations of COD, NHfour +-N and POiv 3−-P in the effluent of MNRC were finer reduced compared to those in the influent, while NHiv +-Due north and POiv iii−-P were concentrated into the recovery solution. Concentrated food ions in the recovery solution could benefit farther recovery of NHiv +-N and PO4 3− -P (for example struvite production)6,34. After the nutrients are recovered, the remaining recovery solution which mainly contains NaCl could be reused in the MNRCs to concentrate NH4 + and PO4 3− in subsequent recovery processes. Thus the recovery solution can be recycled to continuously recover nutrients from wastewater. Information technology was reported that an MFC combined with struvite germination in the anode effluent could produce struvite based on the food ions in wastewater when specific types of wastewater with higher nutrient concentration, such as urine or swine wastewater, were practical35,36. The struvite formation was determined past the concentrations of magnesium, ammonium and phosphate5. Therefore higher concentrations of nutrient ions could promote the struvite product and save magnesium table salt addition. Different from the previous reports, the MNRC could obtain a recovery solution with full-bodied ammonium and phosphate compared to those in the initial wastewater thus the objective wastewater type might be expanded to municipal wastewater.
The internal resistance of a BES is relatively loftier when treating the municipal wastewater with a low conductivity. In the configuration of MNRC, the anode chamber was filled with the granular activated carbon and the cathode chamber was made as thin as possible to minimize the internal resistance. In a BES, buffer solution was always used to improve the conductivity and balance the pH of the electrolyte, while the consumption of buffer solution might increase the operating cost in the practical application. To avoid the improver of buffer, the recirculation mode was chosen to achieve the acid-base neutralization using the H+ and OH− generated by the electrode reactions themselves, which was attempted in a previous work37. Based on the above approaches, the MNRC organisation could run efficiently in the low conductivity status without buffer solution.
The real wastewater contains the charged organic substances. In some previously reported wastewater handling studies based on the BES organization, charged organic molecules can also migrate beyond the ion substitution membranes38,39. Considering the practical application of this organization, an abiotic MNRC structure was operated under an applied electric current of 5 mA to care for the real wastewater sampled from a local sewage treatment found (Gaobeidian, Beijing, Cathay). The concentration of COD in the recovery solution was measured equally ~one mg/Fifty after this experiment. This event indicates that the charged organic fractions (humic acid etc.) in the real domestic wastewater could drift into the recovery solution but in a small-scale amount with the experimental condition of the MNRC organization. The large molecular size and weight of the charged organic molecules might exist the reason for this event. Besides, at relatively low pH of the wastewater and recovery solution (Supporting Information, Fig. S1), the primary species of humic acid and bicarbonate are in molecular forms with neutral accusexl, thus their migration competing with food ions might exist relatively weak. Farther research on real wastewater is needed to investigate the dynamic rest between full-bodied NH4 + and PO4 iii− and other types of ions in the recovery solution during the long-term performance.
The proof-of-concept MNRC has achieved nutrient recovery using the energy contained in the wastewater. However, the concentrations of NH4 + and POiv iii− achieved in the recovery solution were not high enough. Since the current is the driving force of all ions' migration, the electric current generated by the MNRC should be promoted to raise the concentrating and removal of the nutrients. Approaches for increasing the current product include enlarging the scale of the reactor, optimizing the configuration (such as using thinner chambers) and optimizing operation conditions (solution flow rate, etc). Besides, when the objective wastewater contained high concentrations of food ions (for example diluted man urine) and COD, the current output of MNRC was expected to increment and the charge per unit and extent of recovery might be enhanced30. In addition, the volume ratio of the electrolyte and the recovery solution may impact the final N and P concentrations in the recovery solution. This ratio was 2:1 in the present report. When this ratio enlarges, the concentrating results might be enhanced as the nutrient ions could accumulate in a smaller volume of recovery solution. A previous study on a stacked MDC has proved that the smaller volume of solution could accumulate higher concentration of salt41. The effect of the volume ratio on the nutrient removal and recovery performance will exist farther investigated in the future.
The coulombic efficiencies were relatively low (ranging from 7% to fifteen%), indicating that the chemical energy contained in wastewater was not fully utilized in the procedure of producing electricity. Information technology was probably caused past the contact of wastewater with the oxygen in the cathode sleeping accommodation and the COD consumption of microorganisms which did not participate in the electric current product. The result of open up excursion command experiment demonstrates that without current production, well-nigh 66% of COD was consumed by the metabolism of microorganisms (both in the electrode chambers and the tube system) (Supporting Information, Fig. S2). To enhance the coulombic efficiency, future studies will seek to optimize the MNRC configuration and operation mode. The ion transfer process also needs to exist researched to reveal the competitiveness of NHfour +-N and PO4 3−-P with other kinds of ions, to make better use of the electric power by the MNRC and thus produce useful products.
Methods
MNRC construction
Each chamber of an MNRC was a Plexiglas cube, with an inner diameter of 3.0 cm. The widths of these three chambers were 3 cm (anode), 0.5 cm (recovery) and 0.5 cm (cathode) and the effective volumes of these chambers were 21.2 mL (anode), 3.half-dozen mL (recovery) and 3.half-dozen mL (cathode). The anode chamber and the recovery chamber were separated by a cation exchange membrane (CEM, Ultrex CMI7000, Membrane International Inc.). The recovery bedroom and the cathode bedchamber were separated by an anion exchange membrane (AEM, Ultrex AMI-7001, Membrane International Inc.). The anode sleeping room was filled with granular activated carbon (~1 mM in diameter, ~2 to five mM in length, Beijing Chunqiudingsheng Environmental Science and Engineering science Co. Ltd., People's republic of china)42 to agree electrically active biofilms and serve as an anode of the MNRC. A titanium mesh was added in the anode chamber, against the CEM, to serve as an anode current collector. The air cathode was fabricated of carbon cloth (30% wet-proofing) with 0.5 mg/cm2 platinum catalyst and four polytetrafluoroethylene (PTFE) diffusion layers43. A glass fiber separator (ane.0 mM thickness, DC1.0, Jiafu Co., China) was placed against the air cathode44,45. The synthetic wastewater was recirculated from a 100 mL container to the anode bedchamber, then to the cathode sleeping accommodation and back to the container. The tube connecting the anode and cathode chambers was thirty cm in length, as in a previous report37. The recovery solution was continuously circulated between a 50 mL container and the recovery chamber, via a tube ~l cm in length. The apportionment flow rates of electrolyte and recovery solution were kept the same in all experiments.
Microorganisms and medium
The granular activated carbon used in the anode bedroom was inoculated from an MFC operated for six months. The electrolyte was synthetic wastewater that imitated typical domestic wastewater, containing (per liter in deionized water): 0.4 k glucose, 0.020 g NaH2POiv·2H2O, 0.021 g Na2HPO4·12HtwoO, 0.089 thou NH4Cl, 0.016 g NaCl, 0.041 g Na2SO4 and 12.5 mL of trace mineral metals solution46. All experiments were conducted using the same synthetic wastewater solution with measured COD concentration of 369 ± 21 mg/L, measured NH4 +-N concentration of 23.8 ± 1.iii mg/L and measured POiv iii−-P concentration of 6.4 ± 0.half dozen mg/50. The draw solution was 0.164 g/L NaCl solution in well-nigh experiments except as noted.
Experimental procedures
The MNRC anodes were acclimated under MFC fashion (no recovery chamber). A bottle of 100 mL synthetic wastewater was recirculated at a flow rate of fifteen mL/min between the anode and cathode chambers of the MFCs and was renewed every 24 h, which was defined equally an operational bike. After all the MFCs exhibited stable and parallel performance, with repeatable maximum voltage of ~600 mV (1000 Ω) for over x cycles, these MFC reactors were transformed to the MNRC configuration. The MNRCs were operated with 100 mL synthetic wastewater as electrolyte and fifty mL 0.164 g/L NaCl solution equally recovery solution under the same flow charge per unit of 15 mL/min with electrolyte, driven by a peristaltic pump (BT100-1 L, Lange, China). The external resistance of the MNRC was gradually decreased from 1000 Ω to 5 Ω to achieve the peak current, with each resistance used for 3 total cycles47.
When these MNRC reactors could steadily produce current, a preliminary experiment was conducted to optimize flow rates. The MNRCs were operated at menstruum rates of five, 15 and 25 mL/min and achieved the highest current product at 15 mL/min (Supporting Information, Fig. S3). Based on this outcome, subsequent experiments all used the optimal flow rate of 15 mL/min. To investigate the mass remainder of NH4 +-N and PO4 3−-P, an abiotic command (using the MNRC reactor without anode inoculation to operate for 24 h) and an open up circuit command (using the working MNRC to operate in open up circuit for 24 h) experiments were conducted with the flow rate of fifteen mL/min.
As the NaCl contained in recovery solution was used to facilitate internal current conduction, the influence of initial NaCl concentrations on MNRC functioning was likewise investigated. The recovery chamber was supplied with 0, 164 and 328 mg/L NaCl solutions, respectively, to optimize the concentration of recovery solution. The optimal concentration of NaCl solution of 164 mg/50 was used for the residue of the experiments.
To investigate the recovery performance and concentrating extent of ammonium and phosphate, concentrating tests were conducted in which recovery solution was circulated but non replaced during several operational cycles. The pH equally well as concentrations of COD, NH4 +-North and POiv iii−-P in both constructed wastewater and recovery solution were measured at the terminate of each operational cycle (24 h). The concentrating exam was completed subsequently 120 h, when the concentration of ammonium or phosphate in the recovery solution approached peak level. The concentrating tests were repeated and all experiments were conducted in duplicate reactors at room temperature (~25 °C).
Analyses and calculations
A information acquisition system (2700, Keithley Instrument, OH, Usa) was used to monitor the output voltage (U) of MFCs and MNRCs every 20 min throughout the functioning menses. According to Ohm's constabulary, the MNRC current (I) (mA) was calculated every bit I = U/R, in which R was external resistance (Ω).
Concentrations of COD, NH4 +-N and PO4 three−-P were determined by standard methods48. Concentrations of SO4 ii− and Cl− were measured using ion chromatography (ICS-1100, DIONEX, USA). Na+ concentration was measured using inductively coupled plasma atomic emission spectrometry (ICP-AES, IRIS Intrepid Two XSP, Thermo, United states of america). The pH was monitored past a pH meter (Inlab 731, Mettler Toledo, The states).
Theoretical charge demand for a certain ion'southward migration was defined as the electrical charge that was theoretically required for those ions to migrate from electrode chambers to recovery chamber. The full theoretical charge demand was the sum of the theoretical charge demands for all kinds of ions. The total actual charge demand was the total charges passed through the external circuit of the MNRC over an operational cycle, which was calculated as , in which I was the output current. The ratio of total theoretical accuse need to total bodily charge demand was defined as charge transfer efficiency. The ratio of each ion's theoretical charge demand was defined as the charge demand ratio of the MNRC, calculated past:
in which Δ concentration was the concentration increment of a certain kind of ion in the recovery solution; F is Faraday's abiding, 96485 C/mol. In the case of PO4 iii−-P, the boilerplate electrical charge of ions was larger than 1 and smaller than 2, since the primary ionic forms of phosphate were H2POiv − (>80%) and HPO4 2− (<20%) in the solutions in this report. Thus, i.ii was assumed as the boilerplate electric charge of POfour 3−-P ions for calculation and interpretation, although this might introduce some errors.
Additional Information
How to cite this commodity: Chen, 10. et al. Novel Self-driven Microbial Nutrient Recovery Cell with Simultaneous Wastewater Purification. Sci. Rep. v, 15744; doi: 10.1038/srep15744 (2015).
References
-
Shannon, M. A. et al. Science and technology for water purification in the coming decades. Nature 452, 301–310, 10.1038/nature06599 (2008).
-
Johansson Westholm, L. Substrates for phosphorus removal—Potential benefits for on-site wastewater handling? Water Res. 40, 23–36, 10.1016/j.watres.2005.11.006 (2006).
-
Kuzawa, K. et al. Phosphate removal and recovery with a constructed hydrotalcite as an adsorbent. Chemosphere 62, 45–52, 10.1016/j.chemosphere.2005.04.015 (2006).
-
Rittmann, B. E., Mayer, B., Westerhoff, P. & Edwards, M. Capturing the lost phosphorus. Chemosphere 84, 846–853, 10.1016/j.chemosphere.2011.02.001 (2011).
-
Doyle, J. D. & Parsons, S. A. Struvite formation, control and recovery. Water Res. 36, 3925–3940, 10.1016/S0043-1354(02)00126-4 (2002).
-
Le Corre, K. S., Valsami-Jones, E., Hobbs, P. & Parsons, S. A. Phosphorus recovery from wastewater by struvite crystallization: A review. Crit Rev Env Sci Tec. 39, 433–477, 10.1080/10643380701640573 (2009).
-
Lanham, A. B. et al. Metabolic modelling of full-scale enhanced biological phosphorus removal sludge. Water Res. 66, 283–295, 10.1016/j.watres.2014.08.036 (2014).
-
Logan, B. E. et al. Microbial fuel cells: Methodology and engineering. Environ. Sci. Technol. forty, 5181–5192, x.1021/es0605016 (2006).
-
Lovley, D. R. The microbe electric: conversion of organic matter to electricity. Curr. Opin. Biotech. 19, 564–571, 10.1016/j.copbio.2008.ten.005 (2008).
-
Arends, J. B. A. & Verstraete, Due west. 100 years of microbial electricity product: three concepts for the future. Microb. Biotech. 5, 333–346, 10.1111/j.1751-7915.2011.00302.x (2012).
-
Logan, B. Due east. & Rabaey, Thousand. Conversion of Wastes into Bioelectricity and Chemicals by Using Microbial Electrochemical Technologies. Science 337, 686–690, 10.1126/science.1217412 (2012).
-
Wang, H. & Ren, Z. J. A comprehensive review of microbial electrochemical systems as a platform technology. Biotechnology Advances 31, 1796–1807, x.1016/j.biotechadv.2013.10.001 (2013).
-
Li, Westward.-W., Yu, H.-Q. & He, Z. Towards sustainable wastewater treatment by using microbial fuel cells-centered technologies. Energy Environ. Sci. 7, 911–924, 10.1039/C3EE43106A (2014).
-
Davis, J. B. & Yarbrough, H. F. Preliminary experiments on a microbial fuel cell. Science 137, 615–616, 10.1126/science.137.3530.615 (1962).
-
Rabaey, Thousand., Lissens, G., Siciliano, South. D. & Verstraete, W. A microbial fuel jail cell capable of converting glucose to electricity at high charge per unit and efficiency. Biotechnol. Lett. 25, 1531–1535, x.1023/a:1025484009367 (2003).
-
Min, B., Cheng, S. & Logan, B. E. Electricity generation using membrane and table salt bridge microbial fuel cells. Water Res. 39, 1675–1686, ten.1016/j.watres.2005.02.002 (2005).
-
He, Z. & Angenent, 50. T. Application of bacterial biocathodes in microbial fuel cells. Electroanalysis eighteen, 2009–2015, 10.1002/elan.200603628 (2006).
-
Xu, G.-H., Wang, Y.-K., Sheng, K.-P., Mu, Y. & Yu, H.-Q. An MFC-Based Online Monitoring and Alert Organization for Activated Sludge Process. Sci. Rep. 4, 10.1038/srep06779 (2014).
-
Logan, B. East. Extracting hydrogen electricity from renewable resources. Environ. Sci. Technol. 38, 160A–167A, x.1021/es040468s (2004).
-
Hu, H., Fan, Y. & Liu, H. Hydrogen production using single-sleeping accommodation membrane-free microbial electrolysis cells. H2o Res. 42, 4172–4178, x.1016/j.watres.2008.06.015 (2008).
-
Heidrich, E. Southward., Edwards, Due south. R., Dolfing, J., Cotterill, S. E. & Curtis, T. P. Functioning of a pilot scale microbial electrolysis cell fed on domestic wastewater at ambient temperatures for a 12 month period. Bioresour. Technol. 173, 87–95, ten.1016/j.biortech.2014.09.083 (2014).
-
Cao, X. X. et al. A new method for h2o desalination using microbial desalination cells. Environ. Sci. Technol. 43, 7148–7152, ten.1021/es901950j (2009).
-
Mehanna, M. et al. Using microbial desalination cells to reduce water salinity prior to reverse osmosis. Free energy Environ. Sci. three, 1114–1120, 10.1039/c002307h (2010).
-
Jacobson, Thousand. S., Drew, D. M. & He, Z. Efficient salt removal in a continuously operated upflow microbial desalination jail cell with an air cathode. Bioresour. Technol. 102, 376–380, x.1016/j.biortech.2010.06.030 (2011).
-
Morel, A. et al. Microbial desalination cells packed with ion-substitution resin to raise water desalination rate. Bioresour. Technol. 118, 43–48, ten.1016/j.biortech.2012.04.093 (2012).
-
Kim, Y. & Logan, B. E. Simultaneous removal of organic matter and salt ions from saline wastewater in bioelectrochemical systems. Desalination 308, 115–121, 10.1016/j.desal.2012.07.031 (2013).
-
Kelly, P. T. & He, Z. Nutrients removal and recovery in bioelectrochemical systems: A review. Bioresour. Technol. 153, 351–360, 10.1016/j.biortech.2013.12.046 (2014).
-
Cusick, R. D. & Logan, B. E. Phosphate recovery equally struvite within a unmarried bedroom microbial electrolysis cell. Bioresour. Technol. 107, 110–115, 10.1016/j.biortech.2011.12.038 (2012).
-
Cusick, R. D., Ullery, Thousand. L., Dempsey, B. A. & Logan, B. E. Electrochemical struvite precipitation from digestate with a fluidized bed cathode microbial electrolysis cell. Water Enquiry 54, 297–306, 10.1016/j.watres.2014.01.051 (2014).
-
Tice, R. C. & Kim, Y. Free energy efficient reconcentration of diluted human urine using ion exchange membranes in bioelectrochemical systems. Water Enquiry 64, 61–72, 10.1016/j.watres.2014.06.037 (2014).
-
Zhang, F., Li, J. & He, Z. A new method for nutrients removal and recovery from wastewater using a bioelectrochemical system. Bioresour. Technol. 166, 630–634, 10.1016/j.biortech.2014.05.105 (2014).
-
Luo, H., Xu, P., Jenkins, P. Eastward. & Ren, Z. Ionic composition and ship mechanisms in microbial desalination cells. J. Membr. Sci. 409-410, 16–23, x.1016/j.memsci.2012.02.059 (2012).
-
Zuo, K., Yuan, L., Wei, J., Liang, P. & Huang, X. Competitive migration behaviors of multiple ions and their impacts on ion-commutation resin packed microbial desalination cell. Bioresour. Technol. 146, 637–642, x.1016/j.biortech.2013.07.139 (2013).
-
Hao, 10. D., Wang, C. C., van Loosdrecht, Yard. C. G. & Hu, Y. Due south. Looking beyond struvite for P-recovery. Environ. Sci. Technol. 47, 4965–4966, 10.1021/es401140s (2013).
-
Ichihashi, O. & Hirooka, K. Removal and recovery of phosphorus every bit struvite from swine wastewater using microbial fuel prison cell. Bioresour. Technol. 114, 303–307, 10.1016/j.biortech.2012.02.124 (2012).
-
Santoro, C. et al. Ability generation and contaminant removal in unmarried bedroom microbial fuel cells (SCMFCs) treating homo urine. Int J Hydrogen Energ 38, 11543–11551, 10.1016/j.ijhydene.2013.02.070 (2013).
-
Chen, X., Liang, P., Wei, Z. M., Zhang, Ten. Y. & Huang, X. Sustainable water desalination and electricity generation in a separator coupled stacked microbial desalination cell with buffer free electrolyte circulation. Bioresour. Technol. 119, 88–93, ten.1016/j.biortech.2012.05.135 (2012).
-
Forrestal, C., Xu, P. & Ren, Z. Sustainable desalination using a microbial capacitive desalination cell. Energy Environ. Sci. 5, 7161–7167, 10.1039/C2EE21121A (2012).
-
Forrestal, C., Stoll, Z., Xu, P. & Ren, Z. J. Microbial capacitive desalination for integrated organic thing and salt removal and free energy production from unconventional natural gas produced water. Ecology Scientific discipline: Water Enquiry & Engineering 1, 47–55, 10.1039/C4EW00050A (2015).
-
Xiao, G. et al. A systematic analysis of fouling evolution and irreversibility behaviors of MBR supernatant hydrophilic/hydrophobic fractions during microfiltration. J. Membr. Sci. 467, 206–216, 10.1016/j.memsci.2014.05.030 (2014).
-
Zuo, K. et al. A Ten Liter Stacked Microbial Desalination Prison cell Packed With Mixed Ion-Exchange Resins for Secondary Effluent Desalination. Environ. Sci. Technol. 48, 9917–9924, 10.1021/es502075r (2014).
-
Wei, J., Liang, P., Cao, X. & Huang, X. Utilize of inexpensive semicoke and activated carbon as biocathode in microbial fuel cells. Bioresour. Technol. 102, 10431–10435, 10.1016/j.biortech.2011.08.088 (2011).
-
Cheng, Due south., Liu, H. & Logan, B. Eastward. Increased performance of single-chamber microbial fuel cells using an improved cathode structure. Electrochem. Commun. 8, 489–494, ten.1016/j.elecom.2006.01.010 (2006).
-
Zhang, X. Y., Cheng, S. A., Wang, X., Huang, X. & Logan, B. E. Separator characteristics for increasing performance of microbial fuel cells. Environ. Sci. Technol. 43, 8456–8461, 10.1021/es901631p (2009).
-
Zhang, X. Y., Cheng, S. A., Huang, Ten. & Logan, B. E. The use of nylon and glass cobweb filter separators with different pore sizes in air-cathode single-chamber microbial fuel cells. Free energy Environ. Sci. 3, 659–664, 10.1039/b927151a (2010).
-
Lovley, D. R. & Phillips, East. J. P. Novel mode of microbial energy metabolism: organic carbon oxidation coupled to dissimilatory reduction of atomic number 26 or manganese. Appl. Environ. Microbiol. 54, 1472–1480 (1988).
-
Chen, X. et al. Stacked microbial desalination cells to raise water desalination efficiency. Environ. Sci. Technol. 45, 2465–2470, x.1021/es103406m (2011).
-
APHA. Standard Methods for the Examination of Water and Wastewater. 20 edn, American Public Health Association (1998).
Acknowledgements
This enquiry was supported by the Key Program of the National Natural Scientific discipline Foundation of Communist china (No. 51238004) and the Tsinghua Fudaoyuan Enquiry Fund.
Writer information
Affiliations
Contributions
X.C. and D.Due south. carried out the experiments. X.C. analyzed the data and wrote the paper. Ten.H., Ten.Z. and P.Fifty. directed the experiment blueprint, analysis of the data and paper writing. All authors reviewed and approved the manuscript.
Ideals declarations
Competing interests
The authors declare no competing financial interests.
Electronic supplementary material
Rights and permissions
This work is licensed nether a Artistic Commons Attribution four.0 International License. The images or other third party cloth in this article are included in the article'south Creative Commons license, unless indicated otherwise in the credit line; if the material is non included nether the Creative Eatables license, users volition demand to obtain permission from the license holder to reproduce the fabric. To view a copy of this license, visit http://creativecommons.org/licenses/by/4.0/
Reprints and Permissions
About this article
Cite this article
Chen, X., Sun, D., Zhang, X. et al. Novel Self-driven Microbial Food Recovery Cell with Simultaneous Wastewater Purification. Sci Rep 5, 15744 (2015). https://doi.org/10.1038/srep15744
-
Received:
-
Accustomed:
-
Published:
-
DOI : https://doi.org/10.1038/srep15744
Farther reading
Comments
By submitting a comment y'all agree to abide by our Terms and Community Guidelines. If you discover something calumniating or that does non comply with our terms or guidelines delight flag it as inappropriate.
Source: https://www.nature.com/articles/srep15744
0 Response to "Nutrients Removal and Recovery in Bioelectrochemical Systems a Review"
Post a Comment